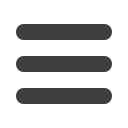
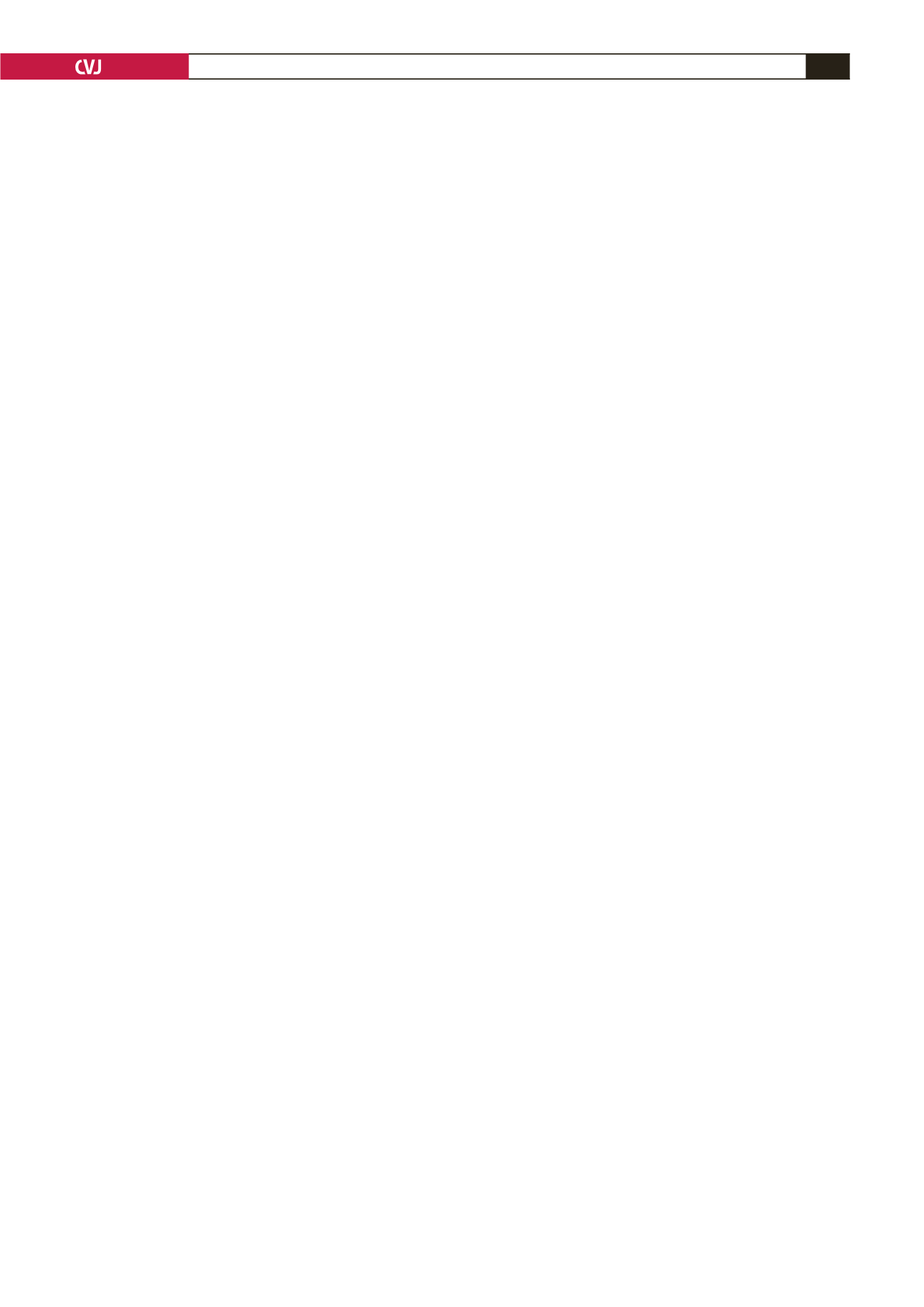
CARDIOVASCULAR JOURNAL OF AFRICA • Volume 27, No 1, January/February 2016
AFRICA
9
these two proteins during normoxic conditions using Co-IP
assays. Therefore, these findings suggest that the induction of
stress is essential to the interaction. Given that hypoxic stress
compromises the integrity of the cellular membranes and
that FLNC has also been shown to interact with the actin
cytoskeleton, it is tempting to speculate that during this time of
cellular stress, the interaction between FLNC and KNCE2 may
be an attempt by the cell to restore cellular membrane integrity,
thereby promoting cellular survival during these conditions.
If this is the case, one could further speculate that mutations
in the genes encoding for KCNE2 or FLNC, or both, which
weaken or abrogate their interaction could result in the cell being
unable to restore membrane integrity, which could lead to acute
myocardial ischaemic arrhythmogenesis. Furthermore, it may
be that hypoxia-induced conformational changes of FLNC are
necessary for the novel KCNE2–FLNC interaction.
During hypoxia, the pattern of co-localisation changed and
the differentiated H9C2 cardiomyocytes showed signs of internal
structural disruption. Both KCNE2 and FLNC displayed
reduced localisation at the surface membrane (Fig. 1i, j, m, n),
while the intracellular co-localisation signal was intensified.
Hypoxic conditions are known to initiate extensive variations in
gene expression, alter protein sub-cellular localisation, and cause
the attenuation of membrane protein translation.
65-67
Furthermore, these findings are consistent with reports that
the HERG
α
-subunit, known to bind KCNE2,
16
showed reduced
membrane localisation during hypoxia.
30,68
The reason for the
observed decrease of these proteins at the membrane requires
further investigation; however, it is interesting to note that both
KCNE2 and filamins have been implicated in processes involving
the internalisation of membrane proteins.
19,69,70
Additionally,
given the evidence of filamins aiding channel localisation,
69,70
and the increase in
KCNE2
gene expression during hypoxia,
31
it would be intriguing to investigate if this interaction serves a
compensatory role to try to restore internally localised channels
to the membrane.
The role of cytoskeletal components, including actin-binding
proteins, in ion channel function and regulatory processes is
a rapidly expanding field of study. Evidence supports their
significant contribution towards channel trafficking and
activity at the plasma membrane itself.
71-73
Furthermore, there
are numerous studies linking the dysfunction of cytoskeletal
proteins with conduction defects and arrhythmias.
72,73
This study is the first to identify the cytoskeletal protein,
FLNC, as a constituent of an ion channel macromolecular
complex, specifically forming part of the KCNE2 interactome.
This observation was only valid during conditions of hypoxia,
although it remains to be seen if other stimuli can elicit the
same association. Together, FLNC and KCNE2 most likely
modulate KCNE2-containing channels, especially pertaining to
their surface expression.
Conclusion
It has long been known that all cells have the ability to
adapt and respond to hypoxic conditions in order to prevent
the harmful effects of oxygen deprivation.
74
In cardiac cells,
potassium channels play a central role in this adaptation.
However, inadequate adaptive responses may lead to serious
cellular damage and cardiac arrhythmias. This has previously
been shown in the cardiovascular system where lack of oxygen
contributes to cardiac arrhythmia.
75,76
This study identified and validated FLNC as an interactor
with KCNE2 under conditions of hypoxia. This finding points
towards new insight and understanding into the mechanisms
in which KCNE2 functions, and could contribute to our
understanding of the interactome in cardiovascular conditions
such as LQTS. Through identification of novel KCNE2
interacting proteins, new genes can be included in searches for
causal and modifying effects of novel arrhythmia disorders.
These findings ultimately advocate intriguing possibilities that
might lead to new therapeutic avenues being discovered.
The KCNE2 Y2H screen was enabled by funding provided by the National
Research Foundation grant FA2006040400017 (VAC). The authors thank
Derick van Vuuren for his assistance with the hypoxic chamber (Division of
Medical Physiology, Stellenbosch University).
References
1.
Schwartz PJ. Prevalence of the congenital long-QT syndrome.
Circulation
2009;
120
(18): 1761–1767.
2.
Schulze Bahr E. Long QT syndromes: genetic basis.
Card Electrophysiol
Clin
2012;
4
(1): 1–16.
3.
Goldenberg I, Zareba W, Moss AJ. Long QT syndrome.
Curr Probl
Cardiol
2008;
33
(11): 629–694.
4.
Schwartz PJ, Crotti L, Insolia R. Long-QT syndrome from genetics to
management.
Circ Arrhythm Electrophysiol
2012;
5
(4): 868–877.
5.
Zipes DP, Wellens HJJ. Sudden cardiac death.
Circulation
1998;
98
(21):
2334–2351.
6.
Bhuiyan MZA, Al-Shahrani S, Al-Aama J, Wilde A, Momenah TS.
Congenital long QT syndrome: an update and present perspective in
Saudi Arabia.
Genet Disord
2013;
1
: 39.
7.
Hedley PL, Jørgensen P, Schlamowitz S,
et al.
The genetic basis of long
QT and short QT syndromes: a mutation update.
Hum Mutat
2009;
30
(11): 1486–1511.
8.
Schwartz PJ, Ackerman MJ, George AL Jr, Wilde AAM. Impact of
genetics on the clinical management of channelopathies.
J Am Coll
Cardiol
2013;
62
(3): 169–180.
9.
Napolitano C. Genetic testing in the long QT syndrome: Development
and validation of an efficient approach to genotyping in clinical practice.
J Am Med Assoc Chic Ill.
2005;
294
(23): 2975–2980.
10. Medeiros-Domingo A, Iturralde-Torres P, Ackerman MJ. Clinical and
genetic characteristics of long QT syndrome.
Rev Esp Cardiol Engl Ed
2007;
60
(7): 739–752.
11. Newton-Cheh C, Eijgelsheim M, Rice KM,
et al
. Common variants at
ten loci influence QT interval duration in the QTGEN Study.
Nat Genet
2009;
41
(4): 399–406.
12. Brink PA, Crotti L, Corfield V,
et al
. Phenotypic variability and unusual
clinical severity of congenital long-QT syndrome in a founder popula-
tion.
Circulation
2005;
112
(17): 2602–2610.
13. Sesti F, Abbott GW, Wei J,
et al.
A common polymorphism associated
with antibiotic-induced cardiac arrhythmia.
Proc Natl Acad Sci
2000;
97
(19): 10613–10618.
14. Sanguinetti MC, Mitcheson JS. Predicting drug–hERG channel inter-
actions that cause acquired long QT syndrome.
Trends Pharmacol Sci
2005;
26
(3): 119–124.
15. Abbott GW, Sesti F, Splawski I,
et al.
MiRP1 forms IKr potassium
channels with HERG and is associated with cardiac arrhythmia.
Cell
1999;
97
(2): 175–187.